Introduction
Literature Review
Methodology
The case study building model
The concrete roof slab
The green roof model
Locations and weather data inputs
Statistical analysis
Results
Seasonal and Climate Effects
Floor effect
Design Parameters Effect
Discussion
Conclusions
Introduction
Green roofs are often indicated as an efficient strategy to mitigate the urban problems in dense cities by reducing urban stormwater runoff as well as decreasing their temperature and, furthermore, guaranteeing building energy savings [1]. Existing studies mainly focused on the cooling potential for urban thermal environments, indicated by factors such as variation of the tree canopy, green roof types, leaf area index (LAI), vegetation configuration (species, layout, and so on), weather conditions (e.g., wind, solar radiation, precipitation), climatic context and building morphology (i.e., high-rise, low-rise); these factors have an increasing significance for global and local warming problems [2]. Energy savings from their use can be observed through the reduced heating and cooling energy consumption required in winter and summer, respectively [3, 4, 5, 6, 7, 8, 9]. In addition, the substrate operates as an effective heat sink to dampen temperature fluctuations. However, under winter conditions or lower temperatures, the subtropical green roofs, unlike temperate latitude studies, can draw the ambient heat upwards into the substrate, increasing heating energy consumption [10].
The building energy saved is greater in single-story structures than in other types of structure [11]. The amount of savings may vary according to the green roof characteristics, including its insulation aspects [12], substrate depth [8], plant selection (height, variation, stomatal resistance, vegetation coverage) [1], leaf area index (LAI) [13], type of irrigation [14], and so on. Many previous studies investigated the influence of the thermal properties of green roofs [10, 13, 15, 16, 17, 18, 19, 20, 21, 22, 23] and their specification details on building energy performance [21, 24, 25, 26, 27, 28].
Green roofs can be classified into three categories, based on their substrate depth, e.g. extensive (0.05-0.20 m), intensive (0.20-2.00 m) or semi-intensive (that presents intermediate characteristics) [3]. Lightweight systems (that is, extensive green roofs) fit perfectly in building retrofitting cases due to their minimal requirements for changes [29]. Therefore, they offer the benefits of easy operation, low maintenance requirements and no-load considerations.
The application of a large-scale, intensive green roof using aromatic plants adapted for low irrigation needs, showed some contributions to decreasing indoor air temperatures and improving thermal comfort conditions, especially in the upper floors of a building that are in direct contact with the green roof. In parallel, the decrease in building energy consumption during the summer and winter periods was shown to be very significant [30]. Likewise, an intensive green roof was able to lower the monthly median overlying air temperature at 300 mm by up to 1°C, in comparison to an adjacent conventional paved roof. The cooling effect was strongest at night, however, it can be reduced when the green roof vegetation is damaged [31]. Furthermore, an intensive green roof contributes to heat and cold storage reduction in the building [32].
The seasonal performance of green roofs and their cooling and heating effectiveness have also been researched in different locations [33, 34, 35]. Indeed, its effectiveness in different regions has been emphasized by some authors, considering that its savings potential is closely related to their characteristics [36] and the climatic conditions of the building location [37]. In some climatic conditions, energy savings potential can be seen despite low vegetation coverage [38, 39]. On the other hand, in other circumstances, green roofs may not be a suitable strategy to foster such savings. Although, in cold climates, green roofs allow good performance (both in summer and winter), in warm climates, they are not advantageous for well-insulated buildings [40].
Although the reduced vegetation coverage minimizes the desired cooling effects, previous studies suggested that green roofs can effectively decrease the indoor temperature if proper maintenance is performed [41, 42, 43, 44, 45]. Likewise, improvements in indoor comfort conditions can also be observed [46]. A reduction in energy demand can be proved by either experimental investigation through field measurements [47, 48, 49, 50, 51, 52] or by numerical simulations [53, 54, 55, 56]. Among other benefits, urban advantages (such as heat mitigation), environmental benefits (such as biodiversity enhancement, carbon dioxide sequestration, air pollution abatement, and improvements to urban livability), and also improvements in city comfort have been revealed by many other studies [57, 58, 59, 60, 61, 62, 63, 64]. Previous studies of green roofs indicated a significant reduction in cooling needs and an influence of the design parameters on performance under different climatic conditions (see Literature Review Section).
LAI and substrate depths, among other elements, play an important role in green roof performance. The influence and benefits of these factors are subject to surrounding climatic conditions. The impact of these variables is generally investigated separately, under a single climate condition or for a few climate scenarios. The joint investigation of these variables and the proof of their impact through statistical methods can provide designers with useful data at a preliminary stage for appropriate green roof selection. The existing studies still present some gaps in practical recommendations under several scenarios, considering the component’s thermal performance to promote wider and better use of green roofs in subtropical and tropical regions. The impact of green roof component variation in energy consumption is sometimes not considered either. Through statistical analyses, the current study, unlike many others, characterizes the significance of these parameters as a priority in the design guideline definitions for an alternative roof. The cooling demand was set as an index, to allow the comparison between different climate conditions. In most cities under subtropical climates, cooling is shown to be a major need. Thus, the cooling energy-saving potential of a concrete roof slab was investigated in comparison to a green roof, characterized by different LAIs and substrate depths for an office building located in distinct subtropical climates. Annual numerical simulations underpin this analysis, which highlights the strategies that provide better thermal performance for the building reference, based on a previously validated green roof model [53]. This study deals with the classic method of green roof investigation by using climatic data. Therefore, the designers can benefit from these initial results, however, in an urban context, urban density, form, and orientation should be considered for the green roof application. In summary, the novelty of this study was to propose an ideal green roof composition, considering a LAI and substrate thickness that could reduce the cooling demand for a commercial building under different climatic conditions.
Literature Review
Table 1 shows the input parameters used in previous studies for green roof vegetation. Although greater levels of relative humidity suggest that the cooling capabilities of green roofs would be reduced under Cfa climatic conditions, Parizotto and Lamberts [18] observed a significant heat gain reduction throughout their experimental study. Therefore, building cooling load reductions were reported by Alexandri and Jones [57], Santamouris et al. [47], and also by Refahi and Talkhabi [8]. The decrease in cooling demand can be influenced by the increase of LAI [8, 13, 65] and lower substrate depth [8]. Thus, the presence of an insulation layer determined a worsening in summer performance and an increased, or at least, similar winter performance compared to the roof reference [66]. The thermal insulation shows a significantly larger influence on heating loads than the thermal properties of the substrates and LAI [65]. It was clearly seen that, as LAI and substrate thickness increased (accompanied by enhanced insulation), the cooling energy savings increased as well [16]. The green roof benefits, in terms of reduction of energy consumption, were reduced to zero on floors not located below it. For retrofitting, the substrate thickness increase from 10 to 15 cm required structural interventions to make its installation possible on existing buildings. Thus, only green roof solutions with a load limit of 1.46 kN/m2 were shown to be suitable for building retrofitting [67]. Green roof performance largely depends on the thermophysical properties of the substrate. In summary, the same plant species combined with different substrate types can achieve heterogeneous performances [68]. These savings can also lead to reductions in CO2, NOx, and SO2 emissions [69]. Besides this, the benefit of extensive green roofs was closely related to climate [37]. Semi-intensive and intensive green roofs lead to lower requirements for cooling energy, compared to extensive solutions [49].
Table 1.
Input parameters used in previous studies for green roof vegetation
References | Location and Köppen-Geiger climate classification | Building typology | Substrate depths (m) and LAI (m²/m²) | Key findings |
[18] | Florianópolis, Brazil (Cfa) | Residential | 0.14 | Bulbine frutescens | Green roof reduced heat gain by 92-97% (warm period) and by 70-84% (cold period) and enhanced the heat loss to 49 and 20% (warm period) and reduced the heat loss by 44 and 52% (cold period) in comparison to ceramic and metallic roofs, respectively |
[57] |
London, UK (Cfb) Montréal, Canada (Dfb) Moscow, Russia (Dfb) Athens, Greece (Csa) Beijing, China (Dwa) Riyadh, Saudi Arabia (BWh) Hong Kong, China (Cfa) Mumbai, India (Aw) Brasília, Brazil (Aw) | Building fabric | - | 5.00 and 10.0m (canyon height) | Cooling demand decrease was great in Hong Kong and Brasília compared to other locations |
[47] | Athens, Greece (Csa) | Nursery school | Mixed Substrate | Local ‘wild’ plants | Cooling load reductions were 6-49% during the summer for the building as a whole and 12-87% for its top floor |
[13] |
La Rochelle, France (Cfb) Athens, Greece (Csa) Stockholm, Sweden (Cfb) | Single-family house | Mixed Substrate | 0.50-5.00 (Sedum) | Cooling demand decreases as the roof insulation level increases (0.00-0.30 m) or as the LAI increases |
[8] |
Bandar Abbas, Iran (BWh) Isfahan, Iran (BWk) Tabriz, Iran (BSk) | Single, two, and three-story residential building | 0.10-0.30 | 0.50-5.00 | Greater cooling decreases were observed at bigger LAI values and lower substrate depth |
[66] | Cosenza, Italy (Csa) | University building | 0.08 | 1.50 (Sedum) | 100% of indoor thermal energy reduction was observed in summer and between 30% to 37% of its outgoing in winter for 3 extensive green roof solutions in comparison to a black bituminous cover |
[67] | Catania, Italy (Csa) | Seven floor multi-story residential building | Variable | 3.00-5.50 (Sedum and Salvia) | The cooling and heating energy consumption decrease was 31-35% and 2-10%, respectively for the top floor of existing buildings retrofitting through different green roof solutions |
[68] | Catania, Italy (Csa) | Test cell (1.35 m × 1.35 m × 1.35 m) | 0.15 | 2.80-5.00 | Greater plant heights, LAI, and leaf reflectivity values improve green roof energy performance in the summer and during the winter the substrate was more significant |
[65] |
Albuquerque, USA (Bsk) Santiago, Chile (Bsk) Melbourne, Australia (Cfb) | Retail stores | 0.15 | 0.10-5.00 | A higher LAI provides a greater reduction in cooling loads due to evapotranspiration of the vegetation-substrate system and canopy’s shading effect. On the other hand, thermal insulation shows a significantly larger influence on heating loads than the thermal properties of substrates and LAI |
[69] |
Nicosia, Cyprus (Csa)
Limassol, Cyprus (Csa) Paphos, Cyprus (Csa) Larnaca, Cyprus (BSh) | Office building | 0.15-0.75 | 1.75-3.50 | The reduction in primary energy consumption was up to 25% and 20% in heating and cooling operations, respectively |
[37] |
Taipei, Taiwan (Cfa) Chiayi, Taiwan (Cwa) | University building | 0.10 | Common local plants | Its cooling effectiveness became more significant when the environmental temperature was higher |
[49] | Lisbon, Portugal (Csa) | Institutional building | 0.10-0.70 | 1.00-5.00 | The semi-intensive and intensive solutions lead to lower requirements for cooling energy: about 36% and 17% of the cooling needs of the extensive solution |
[16] | Orlando, USA (Cfa) | Two-story office building | 0.05-0.70 | 0.001-5.00 | In agreement with the literature, LAI and substrate thickness, accompanied by enhanced insulation, were the most significant parameters for green roof energy performance |
Methodology
The cooling energy-saving potential of two roof designs, that comprise an office building case study, was evaluated through a numerical approach in several Brazilian cities, subject to different climatic conditions. This building model was based on previous studies [25, 70, 71]. Some changes were performed to fit this reference to the Brazilian reality. The main steps of this research consist of: (i) selection of the case study and definition of the building model; (ii) reference model adjustments to represent the concrete slab (building with a concrete roof (CR)); (iii) definition of the green roof parameters and establishment of the building model with the green roof (GR); (iv) definition of the representative cities under different climatic conditions; (v) dynamic energy simulation and extraction of energy consumption data from output files; and (vi) sensitivity analysis using a paired t-test and multivariate factorial analysis and discussion of the results.
The case study building model
In this study, a U.S. Department of Energy (DOE) reference model for an office building was used. Virtual prototyping allows one to emulate real buildings with their real shapes and conditions. It also supports true energy simulation, analyses, and comparison of a whole building or a specific system, which can guide stakeholders’ decision-making [72].
The envelope material may vary according to the building system and its local climate. The thermal property standards for buildings in Brazil only address residential typologies. For this reason, the thermal properties of building walls, roofs, and windows were based on Section 5 of ASHRAE 90.1- 2004 (Energy Standard for Buildings Except Low-Rise Residential Buildings) [73] for each bioclimatic zone in this study. Likewise, this standard was also a reference for the internal loads. However, for this case, considering the great range of this matter (lighting and equipment criteria and so on, for each sort of building), the internal load limits were addressed and revised by DOE laboratories, in partnership with ASHRAE technical committees, industry-specific project guides, and user experience [74]. In order to bring the model closer to reality, HVAC (heating, ventilating, and air conditioning) and other devices were set based on the Commercial Buildings Energy Consumption Survey [75]. ASHRAE 90.1 -2004 [73] parameters were used when applicable. EnergyPlus commercial model files are available on the DOE official website [76] at no cost. Further information can be found in Deru et al. (2011).
A Medium Office DOE model was selected for this study and analyzed using EnergyPlus Version 7.2. Its roof area was approximately 1,660 m² and this reference model consisted of a three-floor multi-story building. This sort of office building stock is quite usual in medium and large cities and has become a UH target by using the vegetated roof strategy. The DOE’s reference file was created for Houston (USA), climate zone 2, according to [73], which belongs to the same group as some Brazilian cities such as Brasília, Porto Alegre, and São Paulo. The building has 15 thermal zones, of which one is a central zone and four are perimeter zones (see Figure 1), totaling 4,982 m² of conditioned area on its three floors. The cooling system is located in the plenum space of every floor that is not conditioned. Three rooftop direct-expansion (DX) and variable air volume (VAV) units were set up for each floor (coefficient of performance - COP = 3.20). According to the default model configuration, which was maintained in this study, the cooling system set point was 24.00°C during office working hours (from 6 a.m. to 10 p.m. on business days and from 6 a.m. to 6 p.m. on Saturdays) and 26.70ºC during non-working hours, Sundays and holidays [72]. Humidity control was not considered; only the temperature of thermal zones was controlled. As a model limitation, the cooling system was the same for all the cities studied in this research.
For additional building information, we have assumed the following values taken from DOE’s model reference (Table 2).
Table 2.
Additional building information
The concrete roof slab
The Laboratory of Energy Efficiency in Buildings (LabEEE) cataloged the thermal properties of walls and roofs commonly used in Brazil [77] . The concrete slab without any cover is one of the most common roofs in Brazil. For this reason, the DOE’s original building model was edited according to LabEEE [77], to represent a typical Brazilian building roof. However, only the cover of the building envelope was edited to minimize handling errors in the validated model created by the DOE. After that, this file was considered to be a conventional model. The following values were assumed for the concrete slab (Table 3).
Table 3.
Concrete slab properties
Property | Value | References |
Surface roughness | Medium | [78] |
Thickness | 0.10 m | [77] |
Thermal conductivity | 1.75 W/m.K | [79] |
Density | 2,200.00 kg/m³ | [79] |
Specific heat | 1,000.00 J/kg.K | [79] |
Thermal absorption considering Kirchhoff’s Law | 0.90 | [79] |
Solar absorptance | 0.70 | [73, 79] |
Thermal transmittance | 3.73 W/m².K | [77] |
The green roof model
Sailor’s EcoRoof model [53] was used to represent the vegetation and substrate layer. The model is a tested and validated EnergyPlus green roof package, and its adoption was due to the possibility of using it with the DOE’s commercial reference building models and to make the initial verification of the results with other studies, such as [25]. Thus, the green roof layer (called RoofVegetation in the EnegyPlus software) was added above the concrete slab in the conventional building model. The EnergyPlus green roof building model was then generated in this manner (see Figure 2). All other characteristics of this model were maintained. We have assumed the following variable values for the EcoRoof parameters: LAI = 2.00; substrate thickness = 0.15 m. For the other parameters, the EnergyPlus default according to [25] was considered. For technical reasons, this roof could be different from a conventional one, but this was not considered in this study to simplify the analysis. In this case, the concrete slab thickness was the same for the vegetated and conventional models. This study was also not intended to represent any specific plants. According to [53], the solar radiation is balanced by sensible (convection) and latent (evaporative) heat flux from the substrate and plant surfaces, combined with the conduction of heat into the substrate. The EnergyPlus numerical simulation was performed by the Conduct Transfer Function Method and the effects of substrate and plants on the green roof energy balance were controlled by the EcoRoof module.
Locations and weather data inputs
For this study, eight cities that are representative of diverse Brazilian climatic conditions were selected, as shown in Figure 3. These cities can be identified by the numbers specified on the map. The Brazilian territory has nine climate areas, of which one (Cwb) was omitted from this study as it was the least representative zone, with only 2.10% occurrence [80] and because no weather data were available in the set weather file format. Table 4 shows the coordinates of the representative cities for the remaining eight climate areas, according to [81].
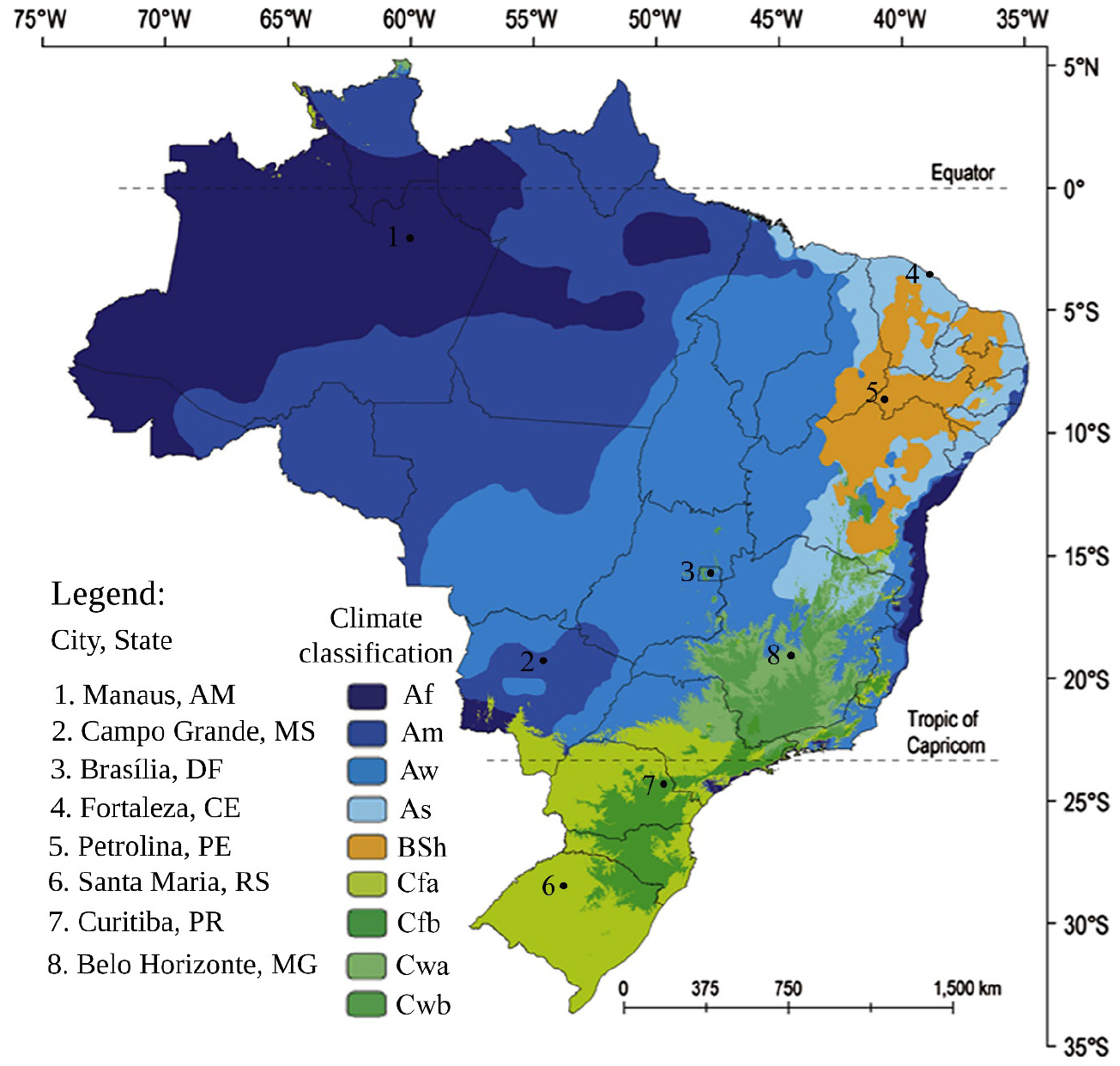
Figure 3.
Location and Köppen-Geiger climate classification of the eight representative Brazilian cities. Source: [80]. Modified by the authors.
Table 4.
Climate classification and coordinates of eight representative Brazilian cities
No. | City, State | Köppen-Geiger Climate Classification | Latitude | Longitude | Elevation (m) | References |
1 | Manaus, AM | Af: Tropical rainforest (without dry season) | 03° 07’ S | 59° 57’ W | 67.00 | [80, 81] |
2 | Campo Grande, MS | Am: Tropical monsoon | 20° 27’ S | 54° 37’ W | 530.70 | [80, 81] |
3 | Brasília, DF | Aw: Tropical savanna with dry winter | 15° 47’ S | 47° 56’ W | 1159.50 | [80, 81, 82] |
4 | Fortaleza, CE | As: Tropical savanna with dry summer | 03° 45’ S | 38° 33’ W | 26.50 | [80, 81] |
5 | Petrolina, PE | BSh: Semi-arid | 09° 22’ S | 40° 28’ W | 370.50 | [80, 81, 82] |
6 | Santa Maria, RS | Cfa: Humid subtropical climate | 29° 42’ S | 53° 42’ W | 95.00 | [80, 81, 82] |
7 | Curitiba, PR | Cfb: Temperate oceanic | 25° 26’ S | 49° 16’ W | 923.50 | [80, 81, 82] |
8 | Belo Horizonte, MG | Cwa: Subtropical highland climate | 19° 56’ S | 43° 56’ W | 915.00 | [81, 82, 83] |
The cooling energy-saving potential of green roofs and concrete roof slabs for office buildings was analyzed in these eight different Brazilian climatic conditions. Typical Meteorological Year (TMY2) weather data files for each city were input in the Energy Plus software. Hourly values of solar radiation and other meteorological parameters are collated from typical months over different years to define the data for a typical year used in this kind of file. The weather data files were specific to each city listed in Table 4 and were derived from the Solar and Wind Energy Resource Assessment (SWERA) project, available on the Energy Plus website [84].
Statistical analysis
Paired t-tests
Monthly energy consumption was verified for different climatic conditions and considered, using paired t-tests (see Table 5). Thus, vegetated-roof efficiency was evaluated in eight Brazilian cities and compared to conventional coverage: Manaus (Af), Campo Grande (Am), Brasília (Aw), Fortaleza (As), Petrolina (Bsh), Santa Maria (Cfa), Curitiba (Cfb) e Belo Horizonte (Cwa). In all cases, efficient performance is considered for a calculated statistical t greater than double the critical value; and null performance, where the statistical t is smaller than double the critical value.
Table 5.
T-paired design for different climatic conditions
In addition to the evaluation of climatic effects, the potential of green roofs to reduce energy consumption was analyzed on different building floors (see Table 6). For this, paired t-tests were used to compare the monthly energy consumption obtained for the first and top floors related to a building model composed of a green roof. Likewise, the first and second-floor energy consumption of conventional and green roof models was compared.
Table 6.
T-paired design for the first and top floor energy consumption
Test | Energy consumption [kWh/(m².month)] | |||||||||||
Jan | Feb | Mar | Apr | May | Jun | Jul | Aug | Sep | Oct | Nov | Dec | |
1st floor | R1 | R2 | R3 | R4 | R5 | R6 | R7 | R8 | R9 | R10 | R11 | R12 |
Top floor | R13 | R14 | R15 | R16 | R17 | R18 | R19 | R20 | R21 | R22 | R23 | R24 |
Two-factor factorial analysis
A two-factor factorial design is a multivariate statistical technique in which data is collected for all possible linear combinations of the levels of the two factors of interest. Each variable of the system under study is considered as a factor and each operating condition of the factors (identified as low or high level) are represented, by convention, as (-) and (+), respectively. Thus, a two-factor factorial analysis performs 2k observations of responses, with k being the number of factors. The effects can be verified as change occurring in the response when changing from a low to a high level and can either be a main effect, obtained by the average difference observed in the response when changing the factor level, or a combined effect, obtained by half the difference between the main effects of one factor at the levels of the other factor.
The effects of the construction parameters of green roofs (more specifically, the influence of substrate layer thickness and LAI) on building energy performance were also evaluated. Two-factorial factor analysis was used for this purpose, making it possible to pinpoint the effect of each factor as well as to identify possible interactions among those factors in building energy performance. In this case, substrate layer thicknesses of 0.05 and 0.30 m and LAIs of 0.50 and 5.00 were evaluated (see Table 7). These limits were defined to describe the different green roof design parameters of this sort of product on the market in Brazil.
Table 7.
Two-factorial factor design
Results
Seasonal and Climate Effects
A seasonal analysis was performed for a building with CR and another with GR, considering the differences in energy consumption for cooling on the top floor in different Brazilian climates, as presented in Figure 4. Besides this, a paired t-test was used (with a significance of 1.00%), as a strategy for statistical comparison between the monthly energy consumption of CR and GR (see Figure 5). The survey aims at detecting the climate types for which the contribution of green cover to reducing the cooling energy consumption was more effective.
Under all climate conditions shown in Figure 4, the total energy consumption for cooling on the top floor was, broadly, lower for the green roof option than for a conventional cover, especially in summer (December-March), in Petrolina (Bsh), Santa Maria (Cfa), and Fortaleza (As). This reduction can also be seen throughout the year, except in Santa Maria (Cfa) and Curitiba (Cfb), during the fall and winter times (May-August). These cities have colder weather and, therefore, lower consumption for cooling in this period. Thus, the positive effect of the green roof was not present in this case. In Curitiba, in some of these months, the total energy consumption for cooling was similar to the conventional one, whereas in Santa Maria the consumption of the green roof was greater. It should be noted that the external effect of a colder climate did not contribute significantly to reducing consumption, considering that the thermal load of people and electrical equipment has a significant weight. The monthly variation of energy consumption on the top floor was also analyzed statistically (see Figure 5).
Using the monthly energy consumption as the response and starting from a paired t-test with 1.00% significance (tcritical = 3.11), it was possible to determine the effect of green covers in Brazilian climates by statistical bias, using conventional covers as the reference. The results suggest two groups: efficient performance (EP), where the calculated t is greater than the critical value; and null performance (NP), where the statistical t is smaller than double the critical value. For the NP group, composed of Curitiba (t-value is 4.14) and Santa Maria (t-value is 3.35), the insertion of green covers did not provide a statistically significant reduction in energy consumption; that is, the energy consumption for the green cover cases and the conventional ones was statistically similar. On the other hand, physically relevant effects are evidenced for t-values greater than 6.22, i.e., twice the t-critical. So, the use of green covers in the EP group, which includes the other cities (Manaus (t-value is 17.02), Belo Horizonte (t-value is 7.61), Brasília (t-value is 9.43), Campo Grande (t-value is 8.14), Petrolina (t-value is 11.11), and Fortaleza (t-value is 33.66)), resulted in a statistically relevant reduction of cooling energy consumption.
Floor effect
The same analysis (paired t-test with 1.00% significance, tcritical = 3.11) was used as a statistical comparison between the CR and GR monthly cooling energy consumption of the first (Figure 6) and second floors (Figure 7) for the office building model. Physically relevant effects are evidenced for t-values greater than 6.22, i.e., twice the t-critical. So, the results pointed to an insignificant statistical effect of GR on the cooling energy consumption of the first and second floors under all climate conditions of the NP group. Besides, in Curitiba (t-value is 0.28) and Santa Maria (t-value is 2.48), the GR did not provide a statistically significant reduction in energy consumption for lower floors, especially in Campo Grande (t-value is 4.81) and Brasília (t-value is 0.69). Although the GR cooling energy savings for the first and second floors have statistical significance in some climates (Manaus (t-value is 7.31), Fortaleza (t-value is 11.70), Petrolina (t-value is 6.56), and Belo Horizonte (t-value is 6.60), the average saving, in terms of kilowatt-hours per square meter per month, was minimal for all cases compared to the top floor results.
Comparing the energy consumed by the first and top floors in each climate, the paired t-test with 1.00% significance (tcritical = 3.11) showed that the statistical treatments are different (tcalc = 11.52). Thus, it is possible to infer that the reduction of energy consumption was restricted to the top floor. So, considering that the first and second floors of a building with green cover do not effectively contribute to reducing energy consumption, hereafter, the monthly energy consumption of the top floor will be used as the simulation response.
Design Parameters Effect
According to Table 7, the simulation results for the annual energy consumption of the top floor for linear combinations of substrate thickness (0.05 and 0.30 m) and LAI value (0.50 and 5.00) were considered for six climatic conditions, as shown in Figure 8. It was found that the cities of Manaus (Af) and Fortaleza (As) presented the highest energy consumption, while Brasilia (Aw) presented the lowest. In all climatic conditions, an increase in LAI (from 0.50 to 5.00) and a decrease in substrate thickness (from 0.30 to 0.05 m) were determinants that enhanced the cooling-energy savings of the top floor. However, when LAI = 5.00 the increase in substrate thickness from 0.05 to 0.30 m (from b to ab) made a less noticeable contribution to reducing the annual energy consumption. On the other hand, the increase of LAI from 0.50 to 5.00 showed a relevant reduction in the annual energy consumption for both tested substrate thicknesses (from (-1) to b and from a to ab). Thus, substrate thickness plays a minor role in building energy savings when the LAI is 5.00 and plays a major role in building energy savings when the LAI is 0.50.
For the purposes of simultaneous study of several variables, separating their effects on the efficiency of the green roof, the statistical technique of factor analysis was used. Thus, the following were studied: the individual effect provided by the substrate thickness (the brown bar in Figure 9), as well as the individual effect of the leaf area index (LAI, the green bar in Figure 9). Complementarily, the combined effect is also evaluated, i.e., the effect of the interaction between LAI and substrate thickness (the gray bar in Figure 9). Thus, the results of factor analyses in Figure 9 are presented in terms of factor loading, i.e., the correlation of the variable with the factor. If this load assumes a positive value, it means that the variable is positively correlated with the factor. If this charge assumes a negative value, this correlation is negative. It is also important to highlight that the greater the factor loading, the greater the item’s contribution to the factor. Thus, it is possible to select which variables have the greatest statistically relevant influence on the efficiency of the green roof.
Figure 9 shows a two factorial factor analysis-synthesis of the reduction in annual cooling energy consumption of the top floor, given in megawatt-hours, and for different Brazilian climates, emphasizing the effect of building parameters (LAI and substrate thickness). It is important to mention that the negative values indicate an increase in energy consumption, while positive ones indicate the opposite. For the analyzed climates (Am, As, Aw, Bsh, and Cwa), the property that was most responsible for reducing the energy consumed by the cooling system was the LAI. The substrate layer thickness had no individual effect, and its combined effects were restricted to the Af and As climates. This means that increasing the substrate layer thickness increases the energy consumption in these climate types. This is due to the thermal inertia provided by the substrate layer, that acts as insulation and delays the heat flux curve during the day (Zhao et al. 2014), making the energy consumption for cooling higher for locations that require cooling in the hours around sunset. In this way, the substrate reduces the heat flux from the interior to the exterior of the building. The decrease in the reduction in cooling energy consumption with increasing substrate layer thickness was verified for some climates in the work of La Roche and Berardi (2014).
Discussion
The results presented in this research confirm the previous studies related to the cooling energy savings of green roofs [8, 13, 16, 18, 37, 47, 49, 57, 65, 66, 67, 68, 69]. For this case study, considering the efficient performance group, the annual cooling reduction on the top floor was 17.70 to 28.80%, when LAI = 2.00 and substrate thickness = 0.15 m, and 31.40 to 39.60%, when LAI = 5.00 and substrate thickness = 0.30 m, compared to conventional covers under Brazilian climates. The potential of the top floor to reduce the cooling energy consumption below a green roof was also noticed [8, 47, 67]. In contrast to the existing literature [8, 13, 16, 37, 49], this study allowed a comparison between the effects of LAI and substrate thickness on green roof performance, although under some climatic conditions, such as those found in Curitiba (Cfb) and Santa Maria (Cfa), the benefits of green roofs were not observed throughout the year but only during the summer. Some authors found benefits of green roofs for the Cfb and Cfa Köppen-Geiger climate classification, such as in Florianópolis, Brazil (Cfa) [18], La Rochelle, France (Cfb) [13], Taipei, Taiwan (Cfa) [37], Orlando, United States (Cfa) [16] and Melbourne, Australia (Cfb) [65]. In this study, the energy consumption for heating was not considered, unlike most other research, that combined both types of analyses (cooling and heating). This could explain the differences in results between researches under those climatic conditions. Besides, an increase in the LAI value had a more significant effect on enhancing the cooling energy savings than an increment in substrate thickness.
To briefly summarize, this research presents the benefits of adopting green roofs to enhance the cooling energy savings and highlights their use as a profitable strategy under some Brazilian climatic conditions. Regarding climate effects, the study allowed the understanding of green roof efficiency for six of the eight climate types studied: Af, Am, Aw, As, Bsh, and Cwa. Considering the representativeness of these climates in the country’s territorial extension, the study suggests the green roof as an interesting solution for reducing cooling energy consumption. It should be considered, however, that the benefits of the green roof in reducing energy consumption were observed only on the top floor of the building. The contributions of the green roof to the reduction of energy consumption for Cw and Cwb climates were only seasonal, and specific studies of the benefits of its use are recommended, when it is implemented in locations with these types of climates. In this case, the authors also recommend considering the energy demand for heating, which is not included in the scope of this study.
The statistical factorial analysis carried out has taken into account not only the cooling needs from the decrease in indoor temperature but also included the influence of the design parameters on performance under different subtropical climatic conditions. The results of this study suggested a general recommendation among the constructive parameters evaluated that could allow an appropriate choice of LAI and substrate thickness relative to the green roof constitution subjected to different climates. Regarding the constructive characteristics, the study demonstrated a greater influence of the leaf area index on the energy efficiency of the green roof for climates Af, Am, Aw, As, Bsh, and Cwa (with a recommended LAI of 5.00). The substrate thickness was presented as a secondary factor in the energy efficiency of the green roof, allowing greater flexibility in the adoption of this solution in existing buildings. Thus, for new buildings, a substrate thickness of 30.00 cm could be used. As for existing buildings, the weight of the substrate layer could make it unfeasible to use the green roof as an energy solution due to structural issues of the building. In this case, a minimum substrate thickness of 5.00 cm is recommended.
Finally, useful information to the building designers concerning the perspectives when adopting a green roof system for an air-conditioned building could provide orientation to its design concept. Thus, the information provided in this paper will be useful to develop Brazilian green roof guidelines for selecting a suitable composition during the design and installation phases. Other cities in the world with a similar Köppen-Geiger climate classification may also benefit from the results of this study.
Conclusions
This study investigated the green roof solution as a design strategy to reduce cooling energy consumption under different Brazilian climatic conditions. Through numerical simulation, thermal energy analysis was carried out for a multi-story office building. Likewise, the influence of green roof design parameters, that present a major contribution to reducing building energy consumption, was evaluated by the statistical technique of factorial analysis.
In this study, the optimal green roof composition was a substrate layer thickness of 30.00 cm and an LAI of 5.00, i.e., the deepest substrate and largest LAI of factor analyses led to the largest reductions in energy consumption. However, especially regarding the reduction in cooling energy consumption, the results suggest that the substrate layer thickness was not as relevant as the LAI under the climatic conditions analyzed. Moreover, a deeper substrate can increase energy consumption for cooling in some climates depending on the operating hours of the air conditioning system. This finding is an important contribution to green roof design, mainly concerning its application over existing buildings slabs. Whereas the substrate layer thickness can be a limiting factor for green roof adoption in building retrofits, due to the considerable additional weight on the existing structural system, the results suggest that the composition of a green roof with a substrate layer thickness of 5.00 cm and an LAI of 5.00 may be the most suitable alternative evaluated in this study.
However, as a limitation of this study, the assumption of a perennial LAI of the green roof species throughout the year, regardless of its seasonal variation under the rainfall and climatic condition in different regions, should be considered. Thus, the obtained results imply the strict and integrated use of irrigation and rainwater harvesting systems to maintain the green roof LAI of the building.
In addition to the construction aspects of the green roof, in terms of substrate layer thickness and LAI, the results suggest that the energy efficiency of this design solution is restricted to the floor immediately below. Therefore, from the economic-financial perspective, it seems that it is more feasible to apply this solution to horizontal buildings than to vertical ones.
The Brazilian climatic variations and their influences on the green roof energy performance were also evaluated. In this regard, considering the annual potential cooling energy savings, it was observed that the green roof did not prove to be efficient for Santa Maria (Cfa) and Curitiba (Cfb), since, in these climates, its energy-saving effects were only observed seasonally.
In summary, this study analyzed the cooling needed to guarantee a baseline for comparison between different climatic conditions over the wide Brazilian territory. Hence, heating needs were not considered in any of the cases. In this case, it seems that the restriction of green roof use in the climates Cfa and Cfb is related to the absence of winter heating systems in the thermo-energetic model analyzed. Besides, further research could be carried out on green roof energy efficiency in Cfa and Cfb Brazilian climates, considering both air conditioning systems, heating, and cooling throughout the year. In a broader sense, the present work contributes to ameliorating the background information and knowledge about the benefits and electric energy savings that green roofs can provide in certain specific Brazilian subtropical climates, in which cooling needs are the highest overall priority.